By: Brittany Kulka
Deep beneath the Earth’s surface, between 660 and 2,900 kilometres down, lies the lower mantle (Fig.1). This region experiences extreme pressures and temperatures. The mineral bridgmanite, which is not only the most abundant mineral in the lower mantle but in the Earth [1], is pivotal for understanding these inaccessible depths. Our understanding of bridgmanite comes from complex scientific techniques, including Diamond Anvil Cell (DAC) X-ray Diffraction (XRD) and Density Functional Theory (DFT) calculations, both central to my DPhil research.
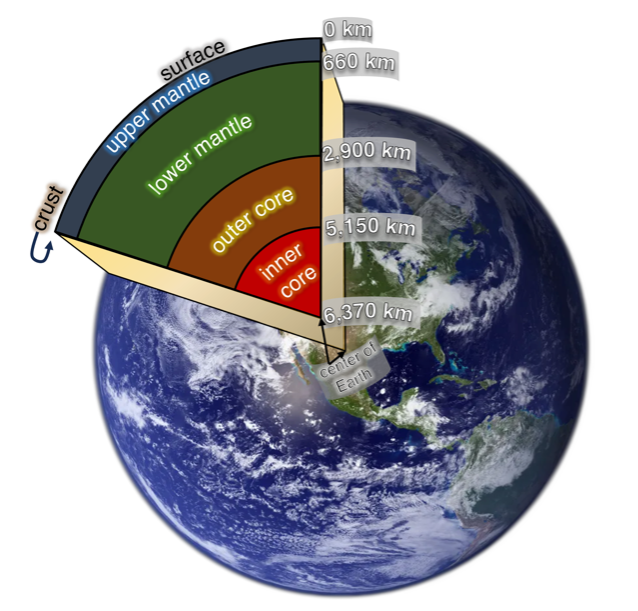
Figure 1. Schematic cross section view of the Earth’s layers and their boundary depths in kilometres (km).
Understanding Bridgmanite: Tools and Techniques
Diamond Anvil Cell (DAC) X-ray Diffraction (XRD)
The DAC technique lets us simulate the extreme conditions of the lower mantle. To do this, we place a sample between two diamonds—our anvils—within a hole that has been drilled into a thin sheet of rhenium metal—a gasket— which supports the sample chamber. As we press the anvils together, we effectively replicate the extreme environment found deep within Earth (Fig.2).
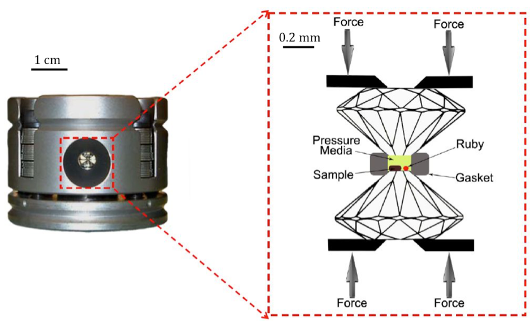
Figure 2. On the left is a DAC. Through the hole on the side is a view of the anvils and the sample chamber showing where a sample is placed for experiments [2].
We collect XRD data using a synchrotron—an apparatus that speeds up electrons to create incredibly bright X-ray light. This allows us to investigate bridgmanite at the atomic scale. In the XRD process, we aim these X-rays at our sample, and as the rays interact with atoms, they scatter in distinctive patterns. We detect these patterns, which tell us about the mineral’s atomic arrangement and how it changes under extreme conditions, giving us a window into the structure of bridgmanite and its high-pressure form, post-perovskite. [3]
Density Functional Theory (DFT) Calculations
DFT is a quantum mechanical modelling method that explores the electronic structures of materials. It helps us understand a material’s properties—such as stability and phase changes—by calculating the behavior of electrons within. In studying bridgmanite, DFT calculations give us an atomic-level view, enhancing our understanding from DAC experiments. This approach predicts how bridgmanite, including its post-perovskite phase, might behave under conditions not easily reproduced in the lab.
Investigating Bridgmanite’s Properties
The inclusion of iron (Fe) and aluminum (Al) alters bridgmanite’s physical attributes, affecting its density and elasticity, which influences seismic wave travel [4,5]. My current research uses in situ XRD experiments at room temperature and high pressures, examining both MgSiO3 and a variant containing Fe and Al bridgmanites. We aim to isolate the pressure effects from thermal effects to better understand these compositions before introducing temperature to experiments through laser-heating. Our findings on how these additions impact bridgmanite’s compressibility and structure uniformity under pressure are significant. They are derived using the third-order Birch-Murnaghan Equation of State, a formula that models how materials compress under pressure to how strong a material is and allows us to predict volumetric and density changes under the Earth’s extreme pressures.
Insights and Implications
Anticipated Findings and Future Directions
Our research highlights intricate behavior of bridgmanite under pressure. Bridgmanite compresses unevenly, exhibiting different responses along its crystal axes—a characteristic known as anisotropy. This uneven compression is illustrated by changes in the crystal’s side lengths, or lattice parameters, with increasing pressure (Fig.3). These changes are intimately connected to the overall volume of the mineral. Understanding this behavior is crucial for interpreting how seismic waves travel through Earth’s lower mantle and offers fresh perspectives on its dynamic processes [6]. Furthermore, bridgmanite’s capacity to integrate different elements informs us about the distribution of those elements within the mantle, advancing our knowledge of Earth’s thermal and chemical makeup. This information is vital for unraveling the history and evolution of the mantle.
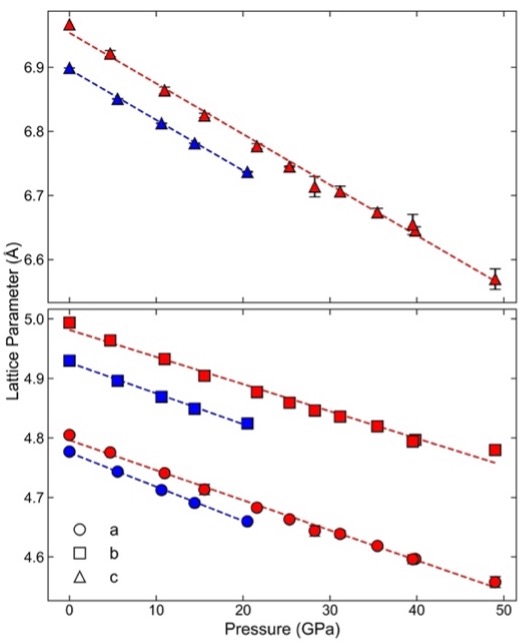
Figure 3. Lattice parameters as a function of pressure for experimental XRD data. Red symbols are for MgSiO3 and blue symbols are for Fe,Al-bearing bridgmanite. The dashed lines are linear fits to the data, illustrating the pressure-dependent decrease in each lattice parameter.
Concluding Remarks
Through DAC (XRD) and DFT calculations, we can uncover bridgmanite’s structural and chemical characteristics. This research enhances our understanding of the lower mantle’s properties and the dynamic interactions of its components. Insights into how bridgmanite structure transitions and how it accommodates different elements and transitions form the basis for interpreting geophysical phenomena and seismic data. As we extend our research to high-temperature conditions, our understanding of the lower mantle will only deepen.
References
[1] Tschauner et al., Science, 346(6213), 1100-1102 (2014).
[2] Dong & Song, Nanowires-Fundamental Research, IntechOpen (2011).
[3] Hirose & Lay, Elements, 4(3), 183-189 (2008).
[4] Li et al., PNAS, 115(8), 1713-1717 (2018).
[5] Kurnosov et al., Nature, 543(7646), 543-546 (2017).
[6] Deschamps et al., Earth Planet. Sci. Lett. 349, 198-208 (2012).